A decades-long endeavor to harness the power of the sun has taken a quantum leap forward.
For the first time ever, scientists have successfully produced a nuclear fusion reaction resulting in a net energy gain, a major milestone that could lead to a source of abundant, inexpensive, and carbon-free power.
Researchers at the National Ignition Facility (NIF) at the Lawrence Livermore National Laboratory in California achieved the breakthrough. The discovery was first reported by the Financial Times on Sunday.
The U.S. Department of Energy is set to announce the milestone today.
Duplicating the nuclear reaction through which energy is created on the sun is considered the “holy grail” of fusion research—a dream scientists have been chasing since the 1950s.
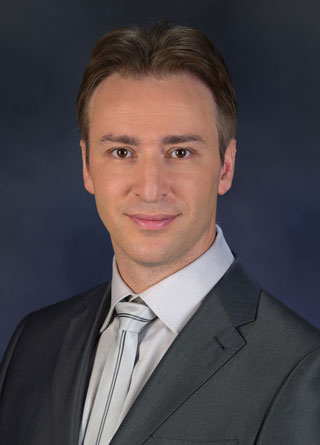
“Clean, plentiful, and economical nuclear fusion is simply the ultimate energy source, and it possesses limitless potential to reshape the future of humankind. But harnessing the energy that fuels the stars presents several engineering challenges that are incredibly hard to overcome,” said Giacomo Po, an assistant professor of mechanical and aerospace engineering at the University of Miami College of Engineering, who is familiar with the work at the NIF and whose own research has applications in nuclear engineering.
Po noted that in recent years, the private sector has invested heavily in fusion energy research. “Several startups are proposing fresh ideas that contribute to the commercial availability of fusion power,” he said.
Po addressed questions about nuclear fusion and what the milestone means.
Scientists have tried for decades to recreate nuclear fusion. Why has it taken so long? What are the obstacles and challenges that have made it so difficult to achieve nuclear fusion?
Tokamaks are the most advanced machines to control nuclear fusion for power generation, and they work by magnetically confining a “burning” deuterium-tritium (DT) plasma. In tokamaks, fusion happens when three plasma parameters reach their critical conditions, namely the plasma density, the plasma temperature, and the confinement time. Reaching such conditions for all three parameters has proved to be extremely challenging. However, clear progress has been made. Earlier this year, for example, the UK-based JET laboratory smashed its own world record in achieving these conditions. The new tokamak under construction in the south of France, the International Thermonuclear Experimental Reactor (ITER), will bring us even closer to attaining commercial fusion energy.
What is unique about the National Ignition Facility at the Lawrence Livermore National Laboratory in Livermore, California, where the nuclear fusion breakthrough was achieved?
The NIF works on a principle known as inertial confinement, which is different from magnetic confinement used in tokamaks. In inertial confinement, fusion is realized by focusing powerful lasers on a small DT fuel pellet, which implodes and creates fusion conditions at its core. The recent breakthrough by NIF is that, for the first time, the output fusion energy was larger than the input laser energy. This is a critical milestone and an exciting accomplishment in inertial confinement fusion, but many technical challenges remain unsolved for this technology.
The machine that generates the reaction undergoes a tremendous amount of heat—something on the order of 10 times hotter than the core of the sun. What material can withstand that kind of heat?
No material can withstand these temperatures. In tokamaks, the trick is that the hot plasma is confined not by structural materials directly, but by a powerful magnetic field that bends the plasma into the shape of a doughnut. However, neutrons produced within the plasma cannot be confined and eventually collide with the structural materials carrying a very high kinetic energy. A “collision cascade” occurs, where the atoms of the structural materials are displaced from their positions in the crystal lattice. Over time, this damages and degrades material. A similar process also happens in fission power plants, which is why they are decommissioned after a certain number of years. But fusion is a much more extreme environment compared to fission, and materials damage remains one of the most severe challenges for the development of commercial fusion reactors.
How is your own research related to nuclear fusion? You have developed novel tools to predict the microstructural evolution of high-temperature and high-strength materials such as refractory metals and ceramics.
To design future commercial fusion reactors, we need to understand the behavior of materials in the fusion environment. However, experimental facilities for materials characterization under fusion conditions are just starting to emerge in the fusion road map. Materials modeling remains one of the fundamental tools to understand how the fusion environment alters the microstructure of materials and eventually degrades their properties. In our research, we develop and use a multiscale computational framework to gain a fundamental understanding of how engineering materials respond in the nuclear fusion environment.
Are you currently working on any research projects that will help to take this breakthrough to the next level?
Embrittlement, segregation, creep, fatigue, swelling, growth, and hydrogen/helium retention are among the most concerning damage modes in structural alloys exposed to fusion neutrons. One of our current projects focuses on understanding the microstructural mechanisms by which irradiation enhances creep damage. We do so by modeling the micro-mechanical interactions between irradiation-induced defect clusters, crystal dislocations, and grain boundaries in engineering alloys. Our research is part of an international effort to overcome arguably the most critical issue in fusion energy—the materials issue.